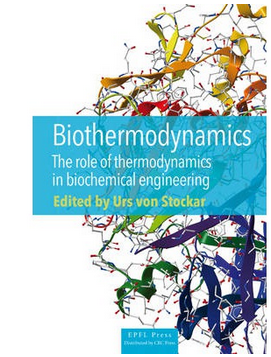
Biothermodynamics : The Role of Thermodynamics in Biochemical Engineering
[BOOK DESCRIPTION]
This book covers the fundamentals of the rapidly growing field of biothermodynamics, showing how thermodynamics can best be applied to applications and processes in biochemical engineering. It describes the rigorous application of thermodynamics in biochemical engineering to rationalize bioprocess development and obviate a substantial fraction of this need for tedious experimental work. As such, this book will appeal to a diverse group of readers, ranging from students and professors in biochemical engineering, to scientists and engineers, for whom it will be a valuable reference.
[TABLE OF CONTENTS]
Part I Fundamentals
1 The Role Of Thermodynamics In Biochemical 3 (10)
Engineering
1.0 Basic remarks on thermodynamics in 3 (2)
biochemical engineering
1.1 Fundamental concepts in equilibrium 5 (1)
thermodynamics
1.2 Charged species, gels and other soft 5 (3)
systems
1.3 Stability and activity of 8 (1)
biomacromolecules
1.4 Thermodynamics of live cells 9 (1)
1.5 Thermodynamic analysis of metabolism 10 (1)
1.6 Conclusions 11 (1)
1.7 References 12 (1)
2 Phase Equilibrium In Non-Electrolyte Systems 13 (12)
2.1 Introduction 13 (1)
2.2 Essential formal relations 14 (5)
2.2.1 Criteria for equilibrium 14 (5)
2.3 Liquid-liquid equilibria 19 (2)
2.4 Solid-liquid equilibria 21 (2)
2.5 References 23 (2)
3 Virial Expansion For Chemical Potentials In 25 (8)
A Dilute Solution For Calculation Of
Liquid-Liquid Equilibria
3.1 Introduction 25 (1)
3.2 Example of protein separation 25 (6)
3.3 References 31 (2)
4 The Thermodynamics Of Electrically Charged 33 (30)
Molecules In Solution
4.1 Why do electrically charged molecules 33 (2)
call for a particular thermodynamic
treatment?
4.2 The thermodynamics of electrolytes 35 (3)
4.2.1 The electrochemical potential 35 (1)
4.2.2 Equilibrium conditions 36 (1)
4.2.3 Appropriate concentration measures 37 (1)
and non-ideality
4.3 Electrostatics 38 (10)
4.3.1 Coulombs law, force of interactions 38 (1)
4.3.2 Short and long range interactions 38 (2)
4.3.3 Simplifications within the 40 (2)
Debye-H?ckel theory
4.3.4 Derivation of the simple 42 (6)
Debye-H?ckel (DH) model
4.4 Empirical and advanced ion activity 48 (8)
coefficient models
4.4.1 Empirical extension of Debye-H?ckel 48 (1)
theory
4.4.2 The Bjerrum theory of ion 49 (2)
association
4.4.3 Modern developments in electrolyte 51 (1)
theory
4.4.4 Pitzer's Model 52 (1)
4.4.5 Guggenheims numerical integration 53 (1)
4.4.6 Integral theory of electrolyte 54 (2)
solutions
4.4 Equations of state for strong 56 (4)
electrolyte thermodynamics
4.5 References 60 (3)
5 Water 63 (16)
5.1 Introduction 63 (1)
5.2 Phenomenological aspects of water 64 (3)
5.3 Molecular properties of water 67 (3)
5.4 Water as a solvent 70 (5)
5.4.1 Electrolytes 71 (1)
5.4.2 Uncharged components 72 (3)
5.5 Further reading 75 (4)
Part II Charged Species, Gels, And Other Soft
Systems
6 Polymers, Polyelectrolytes And Gels 79 (44)
6.1 Flory's Theory of polymer solutions 79 (7)
6.2 Electric Charge on a weak 86 (10)
polyelectrolyte
6.3 Hydrogels: Elementary Equations for 96 (19)
Idealized Networks and Their Swelling
Behavior
6.3.1 Affine Network Model 97 (1)
6.3.2 Phantom Network Model 98 (1)
6.3.3 Swelling theory for hydrogels 98 (2)
6.3.4 Illustration for a perfect 100(4)
tetrafunctional network
6.3.5 Effect of chain entanglement on 104(3)
swelling
6.3.6 Polyelectrolyte hydrogels 107(5)
6.3.7 Hydrogel collapse: phase transition 112(3)
6.4 Appendix: Entropy of mining for polymer 115(6)
solutions
6.5 References 121(2)
7 Self-Assembly Of Amphiphilic Molecules 123(26)
7.1 Introduction 123(1)
7.2 Self-assembly as phase separation 124(4)
7.3 Different types of self-assembled 128(2)
structures
7.4 Aggregation as a "start-stop" process: 130(2)
size and shape of self-assembled structures
7.5 Mass action model for micellization 132(3)
7.6 Factors that influence the critical 135(3)
micelle concentration
7.7 Bilayer structures 138(3)
7.8 Reverse micelles 141(1)
7.9 Microemulsions 142(4)
7.10 Self-assembled structures in 146(1)
applications
7.11 References 147(2)
8 Molecular Thermodynamics Of Partitioning In 149(32)
Aqueous Two-Phase Systems
8.1 Introduction 149(3)
8.2 Flory-Huggins theory applied to aqueous 152(2)
two-phase partition systems
8.3 Dependence of partitioning on system 154(11)
variables
8.3.1 Entropic contribution to the 156(2)
partition coefficient
8.3.2 Enthalpic contribution to the 158(2)
partition coefficient
8.3.3 Relative magnitudes of enthalpic 160(2)
and entropic contributions to partitioning
8.3.4 Effect of polymer molecular mass on 162(1)
partitioning
8.3.5 Effect of tie-line length on 163(2)
partitioning
8.4 Simple interpretation of the effects of 165(7)
added electrolyte
8.4.1 Ion partitioning in systems 166(2)
containing a single salt
8.4.2 Ion partitioning in systems 168(1)
containing a single salt of a
polyelectrolyte with monovalent counter
ions
8.4.3 Protein (biologic solute) 169(3)
partitioning in systems containing a
dominant salt
8.5 Calculation of phase diagrams and 172(3)
partitioning
8.6 Conclusions 175(3)
8.7 References 178(3)
9 Generalization Of Thermodynamic Properties 181(50)
For Selection Of Bioseparation Processes
9.1 Phase behavior in Bioseparation 181(15)
Processes
9.1.1 Phase behavior of 'Bio'-molecules 181(3)
9.1.2 Intermolecular interactions and 184(1)
molecular structure
9.1.3 Physical interactions 184(3)
9.1.4 Chemical interactions 187(1)
9.1.5 Relative strength of molecular 188(1)
interactions
9.1.6 Effect of molecular structure 188(5)
9.1.7 Pure 'bio'-molecules: crystalline 193(3)
and amorphous solids
9.2 Generalized correlation 196(17)
9.2.1 Basic model development 196(2)
9.2.2 Solubilities in mixed solvents 198(4)
9.2.3 Partitioning in mixed solvents and 202(8)
aqueous two phase systems
9.2.4 Sorption in mixed solvents 210(2)
9.2.5 Selectivity of ion exchange resins 212(1)
9.3 Generalized polarity scales 213(2)
9.4 Conclusions 215(1)
9.A Appendix 215(13)
9.A.1 An estimation of log P 215(6)
9.A.2 Pure components and mixtures 221(7)
9.5 References 228(3)
10 Protein Precipitation With Salts And/Or 231(14)
Polymers
10.1 Introduction 231(3)
10.2 Equation of state 234(2)
10.3 The potential of mean force 236(2)
10.4 Precipitation calculations 238(1)
10.5 Generalization to a multicomponent 239(2)
solution
10.6 Crystallization 241(2)
10.7 References 243(2)
11 Multicomponent Ion Exchange Equilibria Of 245(16)
Weak Electrolyte Biomolecules
11.1 Introduction 245(2)
11.2 Multi-component ion exchange of weak 247(3)
electrolytes
11.2.1 Thermodynamic framework 247(2)
11.2.2 The DIX-model for monovalent ions 249(1)
11.3 Experimental case studies 250(6)
11.3.1 Ion exchange of carboxylic and 251(2)
acetyl amino acids
11.3.2 Anion exchange of B-lactam 253(3)
antibiotics
11.4 Conclusions 256(1)
11.5 References 257(4)
Part III Stability And Activity Of
Biomacromolecules
12 Proteins 261(28)
12.1 Introduction 261(1)
12.2 The amino acids in proteins 262(4)
12.3 The three-dimensional structure of 266(5)
protein molecules in aqueous solution
12.4 Non-covalent interactions that 271(8)
determine the structure of a protein
molecule in water
12.4.1 Hydrophobic interaction 272(1)
12.4.2 Electrostatic interactions 273(3)
12.4.3 Dipolar interactions 276(1)
12.4.4 Dispersion interactions 277(1)
12.4.5 Hydrogen bonding 277(2)
12.4.6 Bond lengths and angles 279(1)
12.5 Stability of protein structure in 279(2)
aqueous solution
12.6 Thermodynamic analysis of protein 281(6)
structure stability
12.7 Reversibility of protein denaturation 287(1)
aggregation of unfolded protein molecules
12.8 References 288(1)
13 Thermodynamics In Multiphase Biocatalysis 289(26)
13.1 Why multiphase biocatalysis? 289(1)
13.2 Thermodynamics of enzymatic reactions 290(6)
in aqueous systems
13.3 Non-aqueous media for biocatalysis 296(3)
13.3.1 Fluid phase systems 297(1)
13.3.2 Solid-fluid systems 298(1)
13.4 Using enyzmes in organic solvents 299(4)
13.4.1 Enzyme inactivation in organic 299(1)
solvents
13.4.2 Predicting solvent effects on 300(1)
enzyme stability
13.4.3 Water activity control 301(2)
13.5 Phase equilibria in multiphase 303(7)
enyzmatic reactions
13.5.1 Partition coefficients 303(1)
13.5.2 Aqueous solubilities 304(1)
13.5.3 Calculation of reaction yields at 305(3)
equilibrium
13.5.4 Suspension-to-suspension reactions 308(2)
13.6 Whole cells in organic solvents 310(1)
13.7 List of symbols 311(2)
13.8 References 313(2)
14 Thermodynamics Of The Physical Stability 315(40)
Of Protein Solutions
14.1 Introduction 315(1)
14.2 Factors influencing protein stability 316(13)
14.2.1 Temperature 316(1)
14.2.2 pH effects on protein stability 317(1)
14.2.3 Ligands and co-solutes 318(9)
14.2.4 Salt type and concentration 327(1)
14.2.5 Antimicrobial agents 328(1)
14.2.6 Surfactants 328(1)
14.3 Mechanism of protein aggregation 329(15)
14.3.1 Structural transitions 329(1)
accompanying aggregation
14.3.2 Characterization of the 329(1)
aggregation competent species
14.3.3 Aggregation models, energetics, 330(2)
and rates
14.3.4 Role of conformational stability 332(6)
14.3.5 Role of colloidal stability 338(6)
14.4 Summary and conclusions 344(1)
14.5 References 345(10)
15 Measuring, Interpreting And Modeling The 355(44)
Stabilities And Melting Temperatures Of
B-Form DNAs That Exhibit A Two-State
Helix-To-Coil Transition
15.1 Introduction 355(3)
15.2 Methods for measuring duplex DNA 358(12)
melting thermodynamics
15.2.1 UV absorption spectroscopy 359(6)
15.2.2 Calorimetry 365(5)
15.3 Modeling dsDNA stability and the 370(7)
melting transition
15.3.1 Statistical mechanical models of 370(2)
the melting transition
15.3.2 Linear nearest-neighbor 372(3)
thermodynamic models of B-form DNA
stability and melting
15.3.3 Non-linear NNT models of B-form 375(2)
DNA stability and melting
15.4 Comparing and further improving the 377(10)
performance of NNT models
15.4.1 Duplexes terminating in a 5'-TA 381(1)
group have statistically significant
ΔTm errors
15.4.2 Correcting Tm predictions for 382(2)
duplexes containing 5'-TA type termini
15.4.3 The dependence of B-DNA melting 384(1)
temperatures on ionic strength
15.4.4 Correcting Tm predictions for 385(2)
common features and modifications of
probes and primers
15.5 Final thoughts 387(2)
15.6 References 389(10)
Part IV Thermodynamics In Living Systems
16 Live Cells As Open Non-Equilibrium Systems 399(24)
16.1 Introduction 399(1)
16.2 Balances for open systems 400(5)
16.2.1 General remarks 400(1)
16.2.2 Molar balances 401(1)
16.2.3 Energy balances 402(2)
16.2.4 Entropy balance 404(1)
16.2.5 Gibbs energy balance 404(1)
16.3 Entropy production, forces and fluxes 405(3)
16.3.1 Entropy production in closed 405(1)
systems
16.3.2 Entropy production in non-reactive 406(1)
and reactive flow-systems
16.3.3 Entropy production in steady-state 407(1)
heat conduction
16.3.4 Total entropy production 407(1)
16.4 Flux-force relationships and coupled 408(1)
processes
16.5 The linear energy converter as a model 409(10)
for living systems
16.5.1 Reactions driven against their 409(3)
driving force through coupling
16.5.2 Efficiency of energy converters 412(3)
16.5.3 Driving output reactions up-hill 415(1)
and the principle of minimum entropy
production
16.5.4 Predicting growth kinetics from 416(1)
irreversible thermodynamics
16.5.5 Maintenance as static head 417(2)
situation
16.6 Conclusions 419(2)
16.7 References 421(2)
17 Miniaturization Of Calorimetry: Strengths 423(20)
And Weaknesses For Bioprocess Monitoring And
Control
17.1 Why miniaturization of calorimeters? 423(2)
17.2 Historical roots 425(1)
17.3 Measurement principle 426(6)
17.3.1 Assembly of chip-calorimeter 426(1)
17.3.2 Miniaturization limits 427(3)
17.3.3 Signal evaluation 430(2)
17.4 Calorimetry versus off-gas analysis 432(2)
17.5 Applications of chip-calorimetry 434(4)
17.5.1 Monitoring of discontinuous 434(1)
bioprocesses
17.5.2 Monitoring and control of 435(1)
continuous bioprocesses
17.5.3 Application for biofilm analysis 436(2)
17.6 Outlook 438(1)
17.7 References 438(5)
18 A Thermodynamic Approach To Predict Black 443(32)
Box Model Parameters For Microbial Growth
18.1 Introduction 443(2)
18.2 Catabolic energy production 445(8)
18.2.1 Catabolic Gibbs energy under 445(2)
standard conditions
18.2.2 Catabolic Gibbs energy under 447(5)
non-standard conditions
18.2.3 Threshold inhibition 452(1)
concentrations of catabolic reactants
18.3 Thermodynamic prediction of the 453(8)
parameters in the Herbert-Pirt substrate
distribution relation
18.3.1 The substrate consumption rate for 453(1)
organism maintenance, ms
18.3.2 The biomass reaction substrate 454(4)
parameter, a
18.3.3 The anabolic product reaction 458(2)
substrate parameter b
18.3.4 Stoichiometry of the biomass, 460(1)
product and catabolic reactions
18.4 Prediction of the qp(オ) relationship 461(1)
18.5 Prediction of the process reaction 462(3)
18.5.1 Catabolic products 462(2)
18.5.2 Anabolic products 464(1)
18.5.3 Conclusion 465(1)
18.6 Prediction of the hyperbolic substrate 465(3)
uptake kinetic parameters
18.6.1 The parameters qsmax (or オmax) 465(2)
18.6.2 Affinity, Ks 467(1)
18.6.3 Other mechanisms putting 467(1)
thermodynamically based upper limits on
qs and qp
18.7 Influence of temperature and pH on 468(3)
Black Box model parameters
18.7.1 Effect of temperature 468(1)
18.7.2 Effect of pH 469(2)
18.7.3 Conclusion on temperature and pH- 471(1)
related kinetic effects
18.8 Heat production in biological systems 471(1)
18.9 Conclusion 472(1)
18.10 References 472(1)
18.11 Further reading 472(3)
19 Biothermodynamics Of Live Cells: Energy 475(60)
Dissipation And Heat Generation In Cellular
Cultures
19.1 Why study heat generation and energy 475(2)
dissipation in biotechnology?
19.2 The first law: measuring, interpreting 477(9)
and exploiting heat generation in live
cultures
19.2.1 Applying heat balances to 477(2)
bioreactors and calorimeters
19.2.2 Calorimeters 479(2)
19.2.3 Typical heat generation rates 481(3)
during microbial growth and their
interpretation
19.2.4 On-line monitoring and control of 484(2)
bioprocesses by heat dissipation
measurements
19.3 The second law: energy dissipation, 486(6)
driving force and growth
19.3.1 Energy dissipation and the driving 486(3)
force for growth in chemotrophes
19.3.2 The relationship between the 489(2)
driving force for growth and the biomass
yield in chemotrophes
19.3.3 Summary of the thermodynamics of 491(1)
chemotrophic growth
19.4 Predicting energy and heat dissipation 492(9)
by calculation
19.4.1 Problem statement 492(1)
19.4.2 Standard states 493(1)
19.4.3 Reference states 493(5)
19.4.4 Stoichiometry of the growth 498(3)
reaction and split into catabolic and
biosynthetic reactions
19.5 Results: heat generation and Gibbs 501(11)
energy dissipation as a function of biomass
yield
19.5.1 Aerobic growth 501(1)
19.5.2 Ethanol fermentation 502(2)
19.5.3 Lactic acid fermentation 504(1)
19.5.4 Acetotrophic methanogenesis 505(1)
19.5.5 Autotrophic methanogenesis 506(1)
19.5.6 The relationship between heat 507(1)
generation and free energy dissipation
for chemotrophic growth
19.5.6 Mixotrophic and phototrophic growth 508(4)
19.6 Application: prediction of yield 512(7)
coefficients
19.6.1 Growth efficiency and irreversible 513(1)
thermodynamics
19.6.2 Gibbs energy correlations 514(3)
19.6.3 Product and energy yields for 517(2)
biofuels and biorefineries
19.7 Discussion and conclusions 519(4)
19.A Appendix: Example calculation for 523(8)
prediction of growth stoichiometry
19.A.1 Statement of the problem 523(1)
19.A.2 Thermodynamic data 524(1)
19.A.3 Solution 525(3)
19.A.4 Discussion 528(3)
19.8 References 531(4)
20 Thermodynamic Analysis Of Photosynthesis 535(12)
20.1 Introduction 535(8)
20.2 References 543(4)
Part V Thermodynamics Of Metabolism
21 A Thermodynamic Analysis Of Dicarboxylic 547(34)
Acid Production In Microorganisms
21.1 Introduction 547(1)
21.2 Outline of the approach 548(8)
21.2.1 Black Box thermodynamic analysis 548(1)
of the theoretical dicarboxylic acid
product reaction
21.2.2 Maximal theoretical product yield 549(1)
21.2.3 Stoichiometry of the theoretical 549(3)
product reaction
21.2.4 Alkali consumption, osmotic stress 552(3)
and ionic strength
21.2.5 Thermodynamics of product formation 555(1)
21.3 Thermodynamics of dicarboxylic acid 556(13)
transport
21.3.1 Thermodynamically feasible 556(4)
transport mechanisms
21.3.2 Metabolic energy required for 560(1)
dicarboxylic acid export
21.3.3 Converting Gibbs energy of the 561(1)
theoretical product reaction-into ATP for
growth
21.3.4 Fumaric acid 561(4)
21.3.5 Succinic acid 565(1)
21.3.6 Acid back diffusion 566(3)
21.4 Genetic engineering of target systems 569(1)
based upon thermodynamic analysis results
21.5 Conclusion 569(2)
21.A Appendices 571(6)
21.A.1 Acid/alkali cost 571(1)
21.A.2 Standard ΔfG values 571(1)
21.A.3 In vivo energy aspects of ATP, 572(2)
proton motive force, and fumarate
reductase
21.A.4 Effect of acid back-diffusion on 574(3)
the product yield of dicarboxylic acid
21.6 References 577(4)
22 Thermodynamic Analysis Of Metabolic 581(24)
Pathways
22.1 Introduction 581(1)
22.2 Thermodynamic feasibility analysis of 582(3)
individual metabolic pathways
22.3 Estimation of observable standard 585(9)
Gibbs energies of reaction
22.3.1 K vs. K'-accounting for the 587(1)
non-ideality of the reaction medium
22.3.2 K vs. K''-accounting for the pH 588(6)
and pMg of the reaction medium
22.4 Materials and methods [22] 594(2)
22.4.1 Data for standard Gibbs energies 594(1)
of reaction
22.4.2 Data for physiological conditions 595(1)
in the cytosol
22.4.3 Computational software 596(1)
22.5 Results and discussion 596(4)
23.5.1 Influence of the metabolite 596(4)
concentration range
22.6 Conclusions 600(3)
22.7 References 603(2)
Index 605